Introduction
The formation of 5-aminolevulinic acid (ALA) is a key regulatory step in porphyrin biosynthetic pathway (Beale and Weinstein, 1990; von Wettstein et al., 1995). ALA is synthesized by the ligation of glutamate to tRNAGlu, the reduction of glutamate to glutamate-1-semialdehyde by glutamyl-tRNA reductase (GluTR), and a transamination step by glutamate-1-semialdehyde aminotransferase (GSA). ALA is metabolized to form porphyrins through further reactions. The branch point of the chlorophyll and heme biosynthetic pathway is protoporphyrin IX (Proto IX). The first committed step of chlorophyll biosynthesis is the insertion of Mg2+ into Proto IX (Beale and Weinstein, 1990; von Wettstein et al., 1995). Chlorophylls, one of the end product of porphyrin biosynthetic pathway, play an essential role in photosynthesis by absorbing light and transferring the light energy or electrons to other molecules.
In crop plants, ALA has a variety of agricultural applications as an herbicide (Rebeiz et al., 1984) as well as its ability to confer salt tolerance (Naeem et al., 2010; Watanabe et al., 2000, Zhang et al., 2006) and growth-promoting factor (Hotta et al., 1997a, 1997b; Nguyen et al., 2016; Sasaki et al., 1995). A low concentration of ALA treatment increased chlorophyll synthesis and the growth of plants (Hotta et al., 1997a, 1997b; Memon et al., 2009; Tanaka et al., 1993). ALA application to foliage, soil and root was effective for increasing crop yield (Hotta et al., 1997a, 1997b).
On the other hand, plants treated with a high ALA concentration excessively accumulated photosensitive Proto IX and protochlorophyllide (Pchlide), at the stage preceding chlorophyll (Rebeiz et al., 1984; Tripathy and Chakraborty, 1991), which generate reactive oxygen species (ROS) (Papenbrock and Grimm, 2001; Tanaka and Tanaka, 2007; Tran et al., 2019). The biosynthesis of porphyrin is efficiently regulated at several levels to avoid the accumulation of Proto IX and Pchlide (Papenbrock and Grimm, 2001; Tanaka and Tanaka, 2007). Plants suffer serious photodynamic damage if these control mechanisms are deregulated, for example, in plants with deregulation of porphyrin biosynthetic genes (Jung et al., 2008).
Although the herbicidal properties and growth-promoting effect of ALA in plants are well characterized (Hotta et al., 1997b; Nguyen et al., 2016; Rebeiz et al., 1984), the molecular mechanism of ALA on these physiological actions, particularly a tight regulation of the porphyrin biosynthetic pathway, is little known. In the present study, we focused on the control of the porphyrin biosynthesis at low and high ALA levels to examine the molecular mechanisms of ALA-induced regulation of porphyrin biosynthesis. We analyzed the consequences of low and high ALA treatments on the expression of porphyrin biosynthesis genes, which are critical regulatory steps in the pathway. We have discussed the role of exogenous ALA that modulates porphyrin biosynthesis.
Materials and methods
Plant growth and ALA treatment
The germinated seeds of rice (Oryza sativa cv. Dongjin) plants were sown in pots filled with commercial potting soil and placed under dark condition. After 2 days, rice seedlings were grown in controlled environment plant growth rooms maintained at day/night temperatures of 28℃/25℃ under a 14-h-light/10-h-dark cycle with a 500 µmol m–2 s–1 photosynthetic photon flux density (PPFD). For physiological measurements, 2-week-old rice plants were treated with either a low (50 µM, L-ALA) or a high (500 µM, H-ALA) concentration of ALA with 0.1% Tween-20, when they were at the three-leaf stage. Control plants were treated with water containing 0.1% Tween-20 only. The treated plants were exposed to 14-h-light/10-h-dark cycle for 2 days after 12-h dark incubation following ALA treatment. The second leaves counted from top were taken 18 h and 42 h after ALA treatment for day 1 and day 2 samples, respectively.
Determination of carotenoids, chlorophylls, and porphyrin analysis
Total carotenoids and chlorophylls were extracted with 100% acetone and determined spectrophotometrically according to the method of Lichtenthaler (1987). For measurement of porphyrin content, leaf tissue (0.1 g) was ground in 1 ml of methanol:acetone:0.1 N NaOH (9:10:1) and the homogenate was centrifuged at 10,000 g for 10 min (Lermontova and Grimm, 2000). Porphyrin was separated by HPLC by using Novapak C18 column (4-µm particle size, 4.6×250 mm, Water, Milford, MA, USA) at a flow rate of 1 mL min–1. Porphyrin was eluted with a solvent system of 0.1 M ammonium phosphate (pH 5.8) and methanol. The column elution was monitored by using a fluorescence detector (2474, Waters, Milford, MA, USA) at excitation and emission wavelengths of 400 and 630 nm for Proto IX, 440 and 630 nm for Pchlide, and 415 and 595 nm for Mg-protoporphyrin IX (Mg-Proto IX) and Mg-Proto IX methyester (ME), respectively.
ALA-synthesizing capacity
ALA-synthesizing capacity was measured as described by Papenbrock et al. (1999). Leaf disks were harvested for each sample, incubated in 20 mM phosphate buffer containing 40 mM levulinic acid in the light for 6 h and frozen in liquid nitrogen. Samples were homogenized, resuspended in 1 mL of 20 mM K2HPO4/KH2PO4 (pH 6.9) and centrifuged at 10,000g. The 500 µL supernatant was mixed with 100 µL of ethylacetoacetate, boiled for 10 min, and cooled for 5 min. An equal volume of modified Ehrlichs reagent was added, and the absorption of the chromophore was determined at 553 nm by using the spectrophotometer.
RNA extraction and quantitative reverse transcription (qRT)-PCR
Total RNA was prepared from leaf and stem tissues using TRIzol Reagent (Invitrogen, Carlsbad, CA, USA) in accordance with the manufacturer’s instructions, and 5 μg of RNA from each sample was used for reverse transcription (SuperScript III First-Strand Synthesis System, Invitrogen, Carlsbad, CA, USA). Subsequently, 50 ng of cDNA was used for qRT-PCR analysis, which was carried out with the StepOnePlus™ Real-Time PCR system (Applied Biosystems, Waltham, MA, USA) using Power SYBR™ Green PCR Master Mix (Applied Biosystems, Waltham, MA, USA) and gene-specific primers. The following gene-specific primers were used for PCR amplication: 5’-GCTATGGGTGGTGTTCGACT-3’ and 5’-CGATCTTCTGGAGGCACTTC-3’ for HEMA, 5’-CTCCGTGACTTGACGAAACA-3’ and 5’-GTAGGTTCCAGGCTCCATCA-3’ for GSA, 5’-GTCCACCGTCTCCTTCTCC-3’ and 5’-TGTCAAGTCAAGAGGCCTGA-3’ for ALAD, 5’-ACAGTTCCTCATTGGCCATC-3’ and 5’-CCCATGAAATTTTTGCTGCT-3’ for PPO1, 5’-TGGGACAGCAAAGACAGTGA-3’ and 5’-AAGGCCAGGTTGAAACACAG-3’ for CHLD, 5’-GTGTGGGTTGCGTTCTTTTT-3’ and 5’-GGTGACAATGTGGCTCCTCT-3’ for CHLH, 5’-GTGAATTGCCAGGTTTTCGT-3’ and 5’-GCAATTAGCAAAGCTGCACA-3’ for PORB, and 5’-CTTCATAGGAATGGAAGCTGCGGGTA-3’ and 5’-CGACCACCTTGATCTTCATGCTGCTA-3’ for Actin. The qRT-PCR program consisted of 2 min at 50℃, 10 min at 95℃, and 40 cycles of 15 s at 95℃ and 1 min at 60℃. A melting curve analysis was performed after every PCR reaction to confirm the accuracy of each amplified product. All reactions were set up in triplicate. The control sample was used as a calibrator, with the expression level of the sample set to 1. Actin was used as an internal control.
Results and discussion
Effect of low and high ALA concentrations on photosynthetic pigments in rice plants
ALA plays a role as a control point for providing adequate levels of the common precursor to the chlorophyll and heme branches of porphyrin pathway (Beale and Weinstein, 1990). Exogenous supply of ALA showed contrasting roles as herbicides and growth-promoting factor depending on its concentration in plants (Hotta et al., 1997a, 1997b; Rebeiz et al., 1984; Sasaki et al., 1995). In our previous study, an optimal concentration of ALA increased dry shoot biomass (Nguyen et al., 2016). In the present study, rice plants were treated with either a low (50 µM) or a high (500 µM) concentration of ALA to examine the effect of exogenous ALA on regulation of porphyrin biosynthetic pathway. Foliar application of H-ALA to rice plants developed necrotic symptom on leaves 2 days after the treatment (Fig. 1A). In plants following L-ALA treatment, however, no necrotic symptom was observed on their leaves.
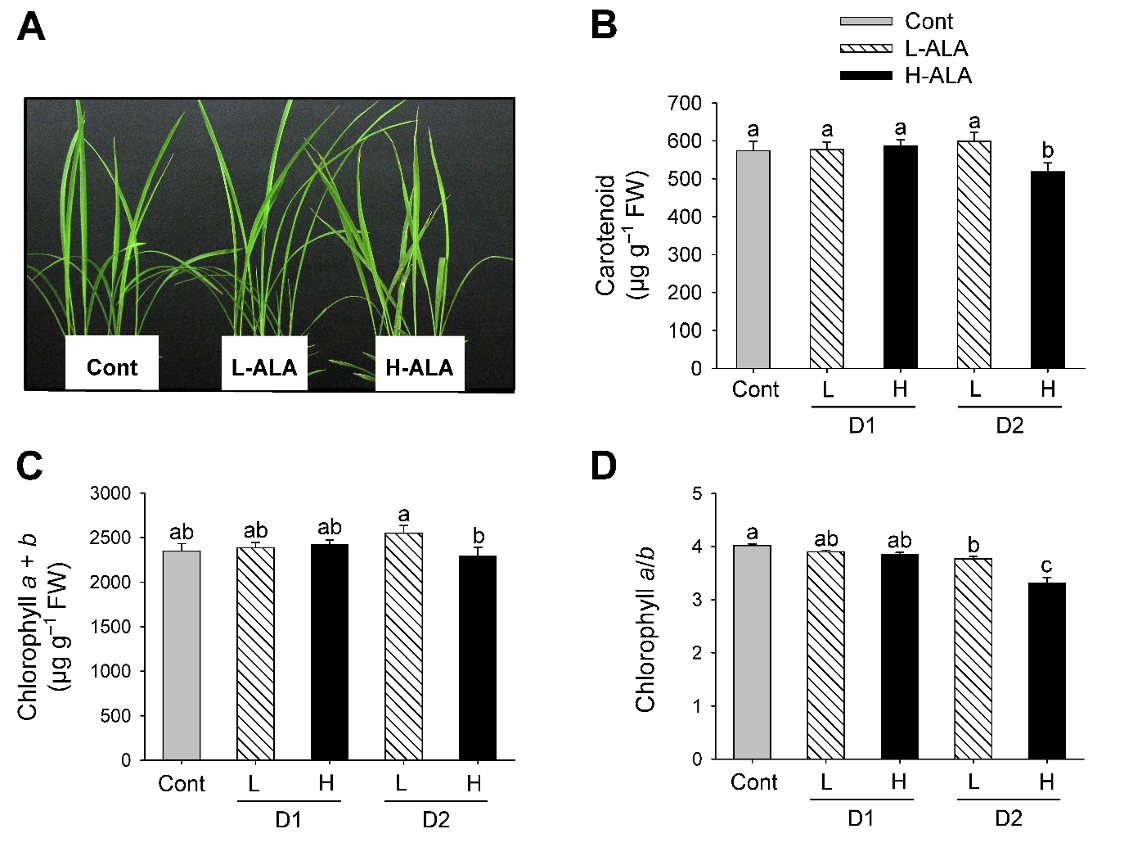
Fig. 1.Effect of exogenous 5-aminolevulinic acid (ALA) treatment on phenotypes and photosynthetic pigments in rice plants. (A) Necrotic phenotype. Photographs were taken 2 days after ALA treatment. (B) Carotenoids. (C) Chlorophyll. (D) Chlorophyll a/b ratio. Rice plants at the three-leaf stage were sprayed with either a low (50 μM) or a high (500 μM) concentration of ALA. Cont: Control (water with 0.1% Tween-20); L-ALA: A low concentration (50 μM) of ALA treatment; H-ALA: A high concentration (500 μM) of ALA treatment; D1: 1 day after ALA treatment; D2: 2 days after ALA treatment. Treatment notations are the same as in Fig. 1. The data represent the mean±S.E. of 6 replicates. a-c: Means denoted by the same letter did not differ significantly at P<0.05 according to Duncan’s multiple range test.
Carotenoids assist in absorbing light energy and protect the photosynthetic apparatus by quenching ROS produced by overexcitation of chlorophylls (Murchie and Niyogi, 2011). Levels of carotenoids significantly decreased 2 days after H-ALA treatment, but they did not change in response to L-ALA treatment, compared to control plants (Fig. 1B). Contents of chlorophyll, the end product of porphyrin biosynthesis, did not differ between L-ALA and H-ALA plants after 1 day of the treatments. Chlorophyll slightly increased in L-ALA plants after 2 days of ALA treatments, but decreased in H-ALA treatment (Fig. 1C). This suggests that exogenous ALA at low concentration may promote chlorophyll synthesis through providing additional chlorophyll precursor, as has been observed previously. ALA treatment stimulated the accumulation of chlorophyll (Hotta et al., 1997a; Memon et al., 2009; Tanaka et al., 1993), and the increased chlorophyll levels in plants enhanced photosynthesis rate (Hotta et al., 1997a, 1997b; Memon et al., 2009). The decreased level of chlorophyll in H-ALA plants (Fig. 1C) could be due to reduced synthesis of chlorophyll or its faster degradation or both. After 2 days of ALA treatment, H-ALA plants showed a greater decrease in chlorophyll a/b ratio than that of L-ALA plants (Fig. 1D). The decrease of chlorophyll a/b ratio was due to a decrease in chlorophyll a content.
Effect of low and high levels of exogenous ALA on the regulation of ALA biosynthesis
We assessed how exogenous ALA at different levels regulates chlorophyll biosynthesis, resulting in alterations in physiological characteristics of plants. First, we examined if exogenous supply of ALA, which is the first committed step of porphyrin biosynthesis (Beale, 2006; Beale and Weinstein, 1990), influences ALA-synthesizing capacity of the treated plants. Plants treated with L-ALA did not exhibit a significant change in ALA-synthesizing capacity compared to untreated controls (Fig. 2). By contrast, plants treated with H-ALA exhibited a great decrease in ALA-synthesizing capacity of rice plants.
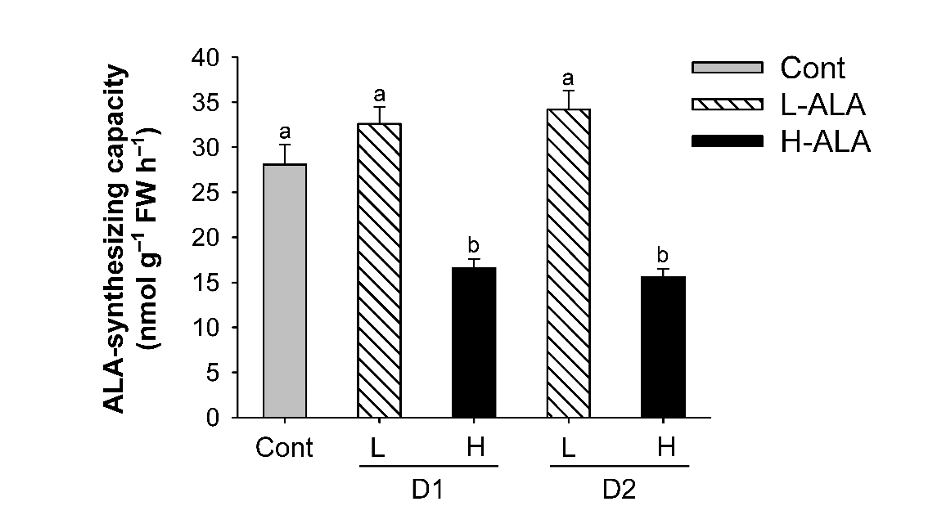
Fig. 2.Effect of exogenous 5-aminolevulinic acid (ALA) treatment on ALA-synthesizing capacity of rice plants. The plants were subjected to the same treatments as in Fig. 1. Treatment notations are the same as in Fig. 1. The data represent the mean±S.E. of 6 replicates. a, b: Means denoted by the same letter did not differ significantly at P<0.05 according to Duncan’s multiple range test.
To examine the ALA-dependent control of the early steps in porphyrin biosynthesis, we analyzed the transcript levels of genes encoding GluTR and GSA, which involve the activity of ALA synthesis (Hedtke et al., 2007), by qRT-PCR. The expression level of HEMA1 encoding GluTR decreased in H-ALA, with a greater decrease 2 days after the treatment (Fig. 3A), which corresponded to the decreased ALA-synthesizing capacity (Fig. 2). Transcript levels of HEMA1 decreased in plants treated with L-ALA, but their levels were higher than those of H-ALA treatment. There was no change in GSA transcript level 1 day after low and high ALA treatments, but decreased 2 days after the treatments, showing that GSA transcript level was less affected by exogenous ALA supply (Fig. 3B). The expression of HEMA1 is regulated by various kinds of stimuli including plastid-derived signals and light, whereas GSA responds only weakly (Cornah et al., 2003; Eckhardt et al., 2004; Tanaka and Tanaka, 2007). Despite the increase in ALA synthesis rate and chlorophyll content, L-ALA plants slightly decreased transcript levels of HEMA1 and GSA (Figs. 1-3). It is possible that post-transcriptional regulation of HEMA2 and GSA in L-ALA plants are to ensure the accompanying increase in ALA-synthesizing capacity that may be required for enhanced chlorophyll biosynthesis upon exogenous ALA. Expression levels of ALAD prominently decreased in H-ALA plants, but remained constant in L-ALA plants (Fig. 3C).
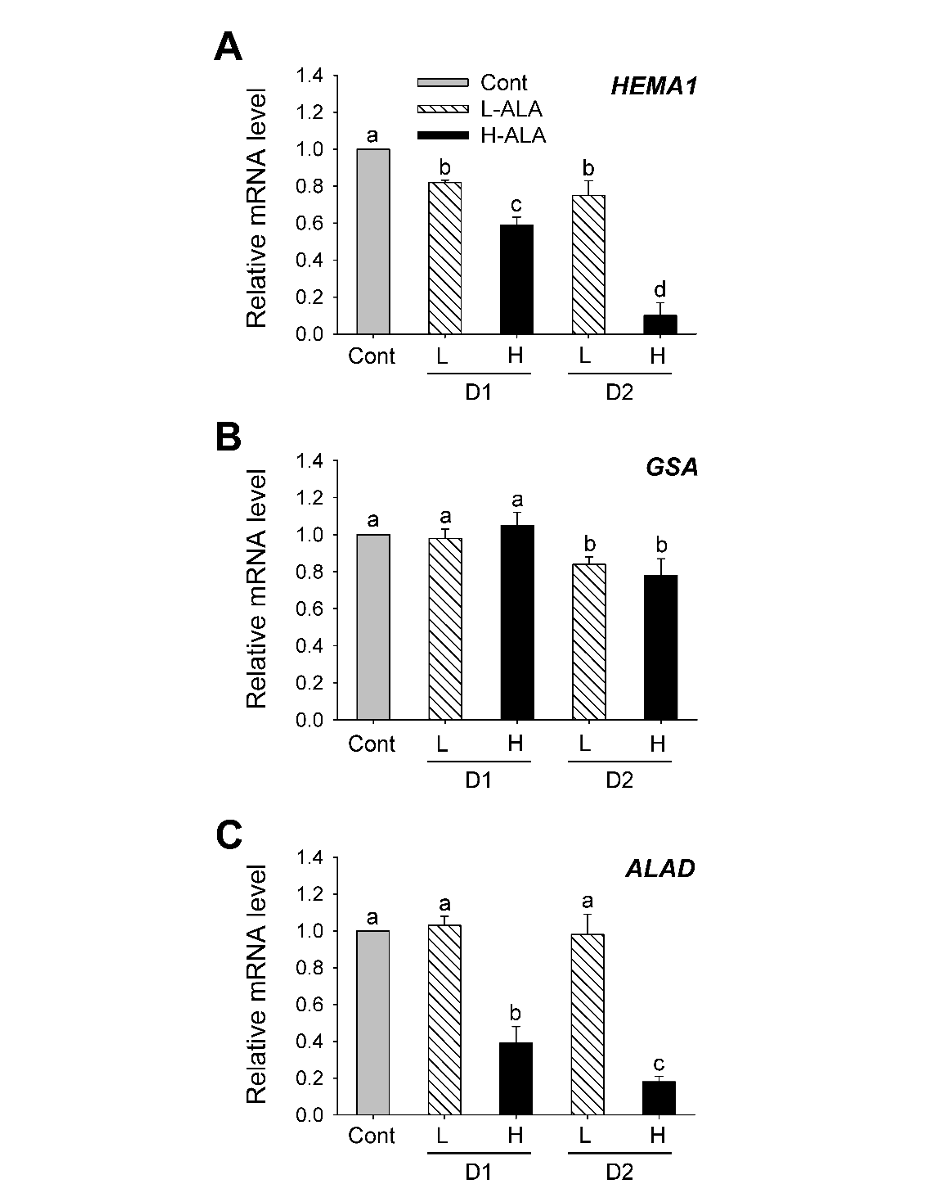
Fig. 3.5-aminolevulinic acid (ALA)-induced changes in the expression of genes encoding porphyrin pathway enzymes involved in ALA metabolism. (A) HEMA1. (B) GSA. (C) ALAD. The plants were subjected to the same treatments as in Fig. 1. Treatment notations are the same as in Fig. 1. Total RNAs were purified from plants and reverse transcribed. The resultant cDNAs were used as templates for qRT-PCR using Actin as an internal control. The control was used for normalization, with the expression level of the sample set to 1. The data represent the mean±S.E. of 6 replicates. a-d: Means denoted by the same letter did not differ significantly at P<0.05 according to Duncan’s multiple range test.
ALA levels differentially regulate the expression of genes involved in chlorophyll biosynthesis
We analyzed the transcript levels of genes encoding enzymes in the chlorophyll biosynthesis after low and high ALA treatments. Plants treated with L-ALA began to increase transcript level of PPO1 encoding protoporphyrinogen oxidase 1 (PPO1) after 2 days of the treatment, whereas plants treated with H-ALA greatly decreased transcript level of PPO1 (Fig. 4A). The expression levels of the genes encoding Mg-chelatase were also examined to characterize the regulation of chlorophyll synthesis. After 1 day of L-ALA treatment, transcript levels of CHLH and CHLD, the gene encoding the Mg-chelatase subunits CHLD and CHLH, remained almost constant in comparison with those of control plants and then slightly decreased after 2 days of the treatment. However, transcript levels of CHLD and CHLH exhibited a marked decrease in plants treated with H-ALA (Fig. 4B and Fig. 4C). Transcript level of PORB encoding Pchlide oxidoreductase B (PORB), which converts Pchlide into chlorophyllide, decreased H-ALA plants, but remained constant in L-ALA plants (Fig. 4D). Our results showed that downregulation of chlorophyll biosynthesis genes at transcript levels were related to necrotic phenotype after 2 days of H-ALA treatment (Fig. 1 and Fig. 4). By contrast, L-ALA plants, which had increased levels of chlorophyll and ALA-synthesizing capacity (Fig. 1 and Fig. 2) sustained transcript levels of porphyrin biosynthesis, thereby retaining sufficient enzymatic capacity for ALA and chlorophyll synthesis. A regulatory coordination between ALA biosynthesis and Mg-porphyrin branch was proposed in transgenic tobacco plants (Alawady and Grimm, 2005).
Our results demonstrate that a regulatory mechanism of porphyrin biosynthetic pathway responded to exogenous supply of ALA by controlling the expression levels of porphyrin biosynthesis genes, particularly rapid downregulation of genes encoding the enzymes in the chlorophyll branch as well as ALA synthesis in plants treated with H-ALA. Tight control of the entire porphyrin biosynthetic pathway is crucial to prevent excessive accumulation of potentially dangerous metabolic intermediates and avoid photodynamic damage. This study has newly characterized the differential molecular mechanism of essential but potentially toxic molecule ALA on the two contrasting physiological processes, plant growth and photodynamic responses. Further studies on ALA-induced regulation of porphyrin biosynthesis are needed before it can be used effectively in agricultural production and weed control.
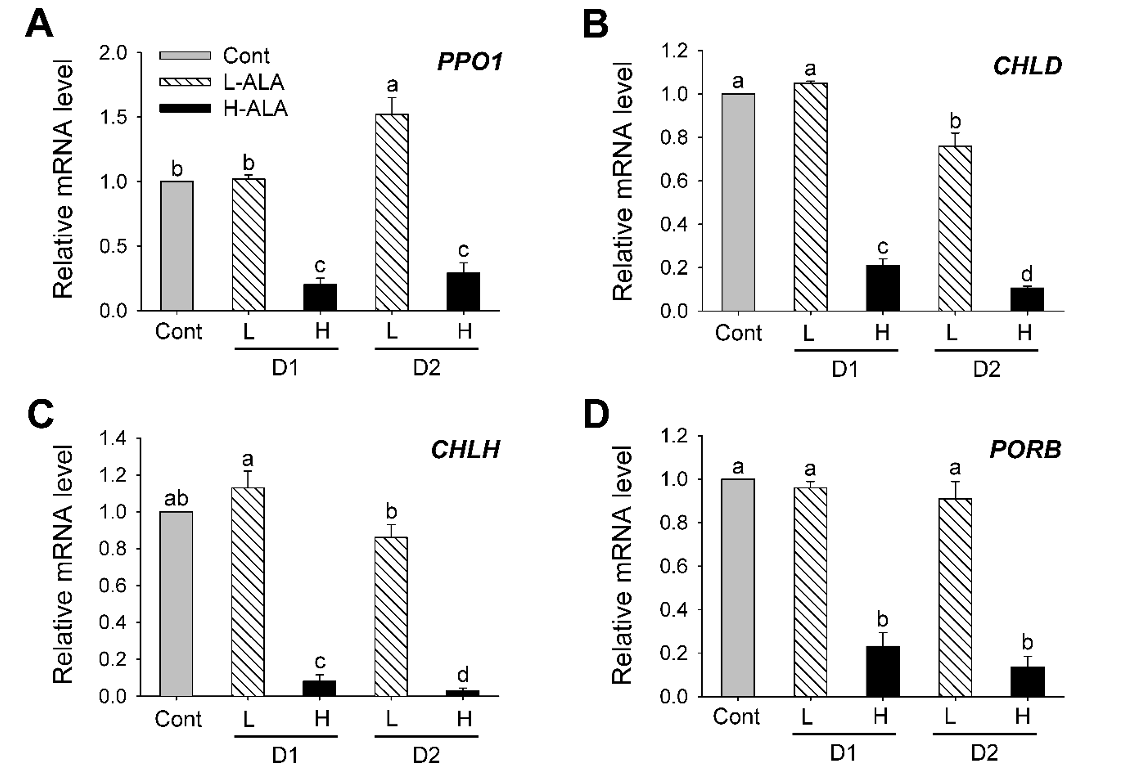
Fig. 4.5-aminolevulinic acid (ALA)-induced changes in the expression of genes encoding porphyrin pathway enzymes in the chlorophyll branch. (A) PPO1. (B) CHLD. (C) CHLH. (C) PORB. The plants were subjected to the same treatments as in Fig. 1. Treatment notations are the same as in Fig. 1. Total RNAs were purified from plants and reverse transcribed. The resultant cDNAs were used as templates for qRT-PCR using Actin as an internal control. The control was used for normalization, with the expression level of the sample set to 1. The data represent the mean±S.E. of 6 replicates. a-d: Means denoted by the same letter did not differ significantly at P < 0.05 according to Duncan’s multiple range test.